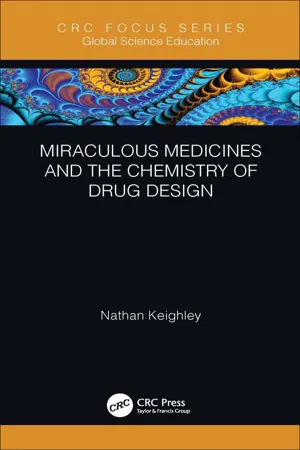
eBook - ePub
Miraculous Medicines and the Chemistry of Drug Design
Nathan Keighley
This is a test
- 126 Seiten
- English
- ePUB (handyfreundlich)
- Über iOS und Android verfügbar
eBook - ePub
Miraculous Medicines and the Chemistry of Drug Design
Nathan Keighley
Angaben zum Buch
Buchvorschau
Inhaltsverzeichnis
Quellenangaben
Über dieses Buch
The subject of chemistry is widely acknowledged as being conceptually challenging, and regarded with a perceived elitism. This book aims to address this dilemma by breaking down the fundamentals of organic chemistry and its importance in medicine, so that readers with any or no background education in chemistry can access the material and gain an appreciation and understanding for the subject. The text is written in a clear and concise manner, using appropriate figures, to explain how the medicine we are so familiar with is designed and produced. Undergraduate students, medical and nursing students, and general audiences will benefit from the accessible format and enjoyable read.
Key Features:
- User-friendly text dealing with the chemical sciences for the non-scientist
- Public understanding of science at the interface of biology and chemistry is in high demand
- The book serves to introduce organic chemistry and its relevance to medicine
- Describes the foundational principles of chemistry without losing the systematic rigor of the subject
Häufig gestellte Fragen
Wie kann ich mein Abo kündigen?
Gehe einfach zum Kontobereich in den Einstellungen und klicke auf „Abo kündigen“ – ganz einfach. Nachdem du gekündigt hast, bleibt deine Mitgliedschaft für den verbleibenden Abozeitraum, den du bereits bezahlt hast, aktiv. Mehr Informationen hier.
(Wie) Kann ich Bücher herunterladen?
Derzeit stehen all unsere auf Mobilgeräte reagierenden ePub-Bücher zum Download über die App zur Verfügung. Die meisten unserer PDFs stehen ebenfalls zum Download bereit; wir arbeiten daran, auch die übrigen PDFs zum Download anzubieten, bei denen dies aktuell noch nicht möglich ist. Weitere Informationen hier.
Welcher Unterschied besteht bei den Preisen zwischen den Aboplänen?
Mit beiden Aboplänen erhältst du vollen Zugang zur Bibliothek und allen Funktionen von Perlego. Die einzigen Unterschiede bestehen im Preis und dem Abozeitraum: Mit dem Jahresabo sparst du auf 12 Monate gerechnet im Vergleich zum Monatsabo rund 30 %.
Was ist Perlego?
Wir sind ein Online-Abodienst für Lehrbücher, bei dem du für weniger als den Preis eines einzelnen Buches pro Monat Zugang zu einer ganzen Online-Bibliothek erhältst. Mit über 1 Million Büchern zu über 1.000 verschiedenen Themen haben wir bestimmt alles, was du brauchst! Weitere Informationen hier.
Unterstützt Perlego Text-zu-Sprache?
Achte auf das Symbol zum Vorlesen in deinem nächsten Buch, um zu sehen, ob du es dir auch anhören kannst. Bei diesem Tool wird dir Text laut vorgelesen, wobei der Text beim Vorlesen auch grafisch hervorgehoben wird. Du kannst das Vorlesen jederzeit anhalten, beschleunigen und verlangsamen. Weitere Informationen hier.
Ist Miraculous Medicines and the Chemistry of Drug Design als Online-PDF/ePub verfügbar?
Ja, du hast Zugang zu Miraculous Medicines and the Chemistry of Drug Design von Nathan Keighley im PDF- und/oder ePub-Format sowie zu anderen beliebten Büchern aus Naturwissenschaften & Organische Chemie. Aus unserem Katalog stehen dir über 1 Million Bücher zur Verfügung.
Information
1 A Brief Background
Life is based on carbon. Organic chemistry is dedicated to this element, whose properties are defined by the nature of the carbon atom. Carbon is in group 4 of the periodic table, which identifies that there are four valence electrons. These are the outer electrons involved in bonding, so a further four electrons are required through bonding to other atoms to satisfy the octet rule; hence, carbon atoms characteristically form four covalent bonds, and each bond comprise of shared pairs of electrons with partner atoms. These may be single bonds, with one shared pair of electrons, or double bonds and even triple bonds, with two and three pairs of electrons being shared. It is common for carbon atoms to bond together in chains and rings to produce the carbon skeleton that defines an organic molecule; the remaining valences are often satisfied by hydrogen atoms, which offer their single electron to form a bonding pair. This produces the simplest class of organic compounds: hydrocarbons, typically separated according to molecular mass through fractional distillation of crude oil.
Other elements from the periodic table, generalised as heteroatoms, are also of importance in organic chemistry. Particularly, nitrogen, oxygen, and the halogens (group 7), and even organometallic species are all vital components of organic chemistry. To fulfil the octet rule, nitrogen, with five valence electrons, requires three more electrons, so typically it forms three bonds; oxygen (group 6) needs two electrons, so tends to form two bonds; while the halogens require one more electron, so form single bonds. The valence electrons govern the chemistry of the elements, and so are the most important. However, they do not represent the entirety of the atoms electron configuration; it is worth noting that the total number of electrons, whose charge is fundamentally negative, equals the atomic number, Z, which is the number of positively charged protons within the atomic nucleus to give a neutral atom. The remainder of the atomic mass, which for carbon is 12 g mol‒1, is made up with neutrons in other words the carbon nucleus is composed of six protons and six neutrons. The number of neutrons may vary to give isotopes, which have a different atomic mass but the same chemical properties, since it is the valence electrons that govern chemistry. The appropriate number of valence electrons may be added or subtracted to give charged atoms, called ions.
The number of covalent bonds that an atom forms governs molecular geometry. This is explained by valence-shell electron pair repulsion (VSEPR) theory, which predicts that pairs of electrons will occupy positions around the atom as far apart from one another as possible. This leads to characteristic molecular shapes. For a carbon atom within a molecule, with four equivalent bonding pairs of electrons, each bonding pair will be positioned equidistantly in three-dimensional space to produce a tetrahedral geometry, with equal 109.5 degree angles between bonds. For a boron atom forming three equivalent bonds, the bonding pairs of electrons will be separated the maximum distance apart to produce a trigonal planar shape, with 120 degree bond angles. Scenarios where multiple bonding is present result in different molecular shapes. If the carbon atom was to have a double bond with one of its neighbours, as in the case of ethene, there will be three points of electron density and, consequently, the molecule will adopt a trigonal planar shape. However, the electron density is not evenly distributed around the carbon atom; more of it is present in the double bond than in the two single bonds, and, therefore, the shape will be slightly distorted. VSEPR theory predicts that electrons that are not involved in bonding, or ‘lone pairs’, will repel more and influence molecular shape. For example, although a nitrogen may have three bonds similar to boron, the presence of a lone pair pushes the bonding electron pairs away to produce a trigonal pyramidal shape. Likewise, water molecules are not linear; the presence of two lone pairs pushes the oxygen-hydrogen bonds down into a V-shape. The shapes of molecules, dependent on the nature of the atoms and their bonding, have a dramatic effect on chemistry because molecular geometry influences how reactions proceed.
Reactions of organic molecules are, for the most part, governed by the presence of heteroatoms. They have the ability to disturb the electron density within the local area of the hydrocarbon skeleton and therefore create a reactive centre. The positioning and nature of the bonding of heteroatoms in organic molecules are identified as functional groups, which will undergo characteristic reactions.
Understanding electrons is essential to chemistry. In a reaction, chemical bonds must be broken: this may be a heterolytic cleavage, where two electrons in the bond move to one species to form ions, or a homolytic cleavage, where the pair of electrons are shared to produce free radicals. In organic chemistry, the movement of electrons is shown with curly arrows to produce organic reaction mechanisms, which will feature later in the text. Since reactivity is the movement of electrons to break weak bonds and make new, stronger bonds, it is possible to predict how an organic reaction mechanism will proceed. For two reacting molecules, identify where the electrons are coming from. This molecule is termed the nucleophile—a negatively charged ion, or neutral molecule with a lone pair of electrons which are donated to form a covalent bond. The electrons are received by the electron-deficient molecule called an electrophile. Whether a given molecule will react as a nucleophile or an electrophile depends on the functional groups that are present.
Organic reactions can be classified as either acid-base reactions or redox reactions. The transfer of a hydrogen ion (a proton) identifies an acid-base reaction, while a change in functional group from reactants to products shows reduction-oxidation reactions. Processes that involve homolytic bond cleavage are called radical reactions. Processes that involve heterolytic bond breaking are called polar reactions. Polar reactions are the most common type of reaction of organic molecules and involve reactions between polar molecules and/or ions. There are three main classes of polar reactions. An addition reaction involves the combining of two molecules to yield a single product, while in an elimination reaction, one reactant molecule is converted into two product molecules. In a substitution reaction, one functional group on the molecule is replaced by another.
Two organic molecules can be composed of the exact same proportions of atoms in other words have the same molecular formula, but the way in which the atoms are arranged may be completely different to give two unique molecules. These are termed isomers. There are three different types of structural isomerism. The functional group may be placed at different positions on the carbon chain in positional isomers, or the atoms may be arranged in such a way to give a different functional group, known as functional group isomerism. Equally, it can be the hydrocarbon chain itself that is arranged differently to give different chain isomers.
Figure 1.1 demonstrates how atoms can be arranged to give different molecules from the same molecular formula. Note that the way in which these molecules are drawn is the skeletal formula. Each corner represents a carbon atom; only important heteroatoms are labelled and the remaining valences of the carbon atoms are bonds to hydrogen atoms (not drawn to save convolution). It can be clearly seen that three of the molecules are alcohols (OH) and molecule (c) is a different functional group. The position of the OH is different between molecules (a) and (b), while in molecule (c), the carbon chain has branched. Each of these molecules will have different properties and reactivity.

FIGURE 1.1 Structural Isomers Possible for the Molecular Formula C4H10O.
The way in which these molecules are drawn does not portray their three-dimensional structure, which is important because molecules with the same structural formula can be arranged differently in space to produce stereoisomerism. Molecules are continuously moving and vibrating and rotation around single bonds, which means that they can adopt different conformations. Molecules with the same structure can also exhibit different configurations, where they may exist as non-superimposable mirror images, or groups of atoms may be held in different spatial arrangements on either side of a rigid carbon-carbon double bond. This concept may be difficult to envisage, but is crucially important in drug design, as will be seen later in the text. For example, the unfortunate consequences of thalidomide, used for morning sickness, was due to the drug been administered as a fifty-fifty mixture of the mirror images, where one of the spatial arrangements caused harm.
Organic reactions are used to synthesise drugs. Considerations of the stereochemistry are obviously vital in the design of new medicines. In cases where one of the two stereoisomers is the active drug, an asymmetric synthesis is required where special measures are taken to ensure stereospecificity. With knowledge of the characteristic reactions that different functional groups display, organic chemists can synthesise a target drug molecule from the relevant readily available starting materials. To build a target molecule, making carbon-carbon bonds is essential. Functional groups that will undergo addition reactions are useful for this purpose. To ensure strong interactions with the drugs’ biological target, a particular functional group may be needed in the molecule. Here, a substitution reaction may be relevant. Ultimately, a drug molecule is made with the correct size, shape, correctly positioned functional groups, and chemical properties that will interact with the biological system to produce a biological response. A selection of functional groups that are key to organic synthesis are shown in Figure 1.2. Whether the compound acts as a medicine or a poison depends on the dose level of the compound. This can be described by the drugs therapeutic index, which is a measure of a drugs beneficial effect at low dose versus its harmful effects at high dose. No drug is absolutely harmless and drugs may vary in the side effects they have.
The design of a medicinally useful compound is a long and arduous process. The first difficulty is identifying a biological target for which a therapeutic molecule can be designed that will interact in such a way as to combat the disease. Understanding the molecular basis of disease is paramount in order to be able to design a compound with the correct chemical structure to not only bind to the biological target, but also interact in a way that produces a biological response that suppresses the malfunctions caused by the disease that have an adverse effect on good health. Once a target is identified and a target molecule determined through computational analysis, organic chemists strive to synthesise this lead compound. Many slightly differing structural analogues of the lead compound may need to be produced to optimise the properties of the drug. This includes minimising side effects. After a series of clinical trials, and billions of dollars of investment, the drug may become available to market.

FIGURE 1.2 A List of Selected Functional Groups Where R = the Rest of a Molecule and X = Halogen Atoms in other words F, Cl, Br, and I.
The work undertaken by medicinal chemists has been instrumental in the improvements observed in the health of society and the increase in life expectancy in modern times. Surgical procedures that are now routine, prior to the development of antibiotics, carried great risk of septicaemia and death. Many diseases caused by harmful pathogens can now be treated. Malfunctions of the body or mind that are understood on a molecular basis can also be cured by medicines. Conditions that were once life-threatening, such as diabetes or heart disease, now have drugs that are available to preserve a healthy life. As people are now living longer, society is faced with the challenges of an aging population. Diseases such as cancer and neurological deterioration in Alzheimer’s and dementia are now starting to be understood and drugs, which strive to combat these diseases, are available on the market. It is important to acknowledge how much chemistry has improved our lives.
2 Finding a Target
THE CELL: NATURE’S LABORATORY
Drugs must interact with the body to produce a biological response. On a microscopic level, the tissues which comprise our bodies are made up of individual cells. It is with the cells that drugs perform their function. The cell is a very complex structure and offers numerous targets on which drugs can work. The cell likewise can be considered simply as a reaction vessel; living creatures are composed of chemicals and are obedient to the same laws of chemistry as any laboratory experiment. Knowledge of the principles of chemistry means that medicinal chemists can predict how molecules will interact with a biological target and design drugs that will generate the required response to alleviate the symptoms of a disease. The cell contains thousands of essential molecules, where chemical energy drives their biosynthetic reactions to produce the cell’s fundamental components. Small molecules, which are predominantly obtained from the diet, are used to synthesise the giant macromolecules of the cell. Principles of thermodynamics give order to these polymers in this chemical system resulting in them adopting predetermined conformations, dependent on the sequence of their monomer sub-units. These macromolecules assemble to form the vital structures of the cell, such as receptors, transport proteins, enzymes, as well as non-protein structures such as plasma membranes and nucleic acids. It is within this ensemble of molecules that a molecular target for a particular disease must be identified and a drug designed to interact with this target in such a way as to serve as a therapy.
The elements that are prevalent in organic chemistry are indeed the fundamental building blocks of the cell also. Nature carries out its organic reactions within the aqueous environment inside the cell, with water serving as a solvent. Polar molecules are dissolved; those with functional groups containing electronegative atoms that pull the electron density of the molecule towards themselves are held within the aqueous medium, while non-polar hydrophobic compounds remain separate from the internal cellular solution, known as the cytoplasm. This property of different solubility of biological molecules is crucial to the cell, for it governs how molecules interact and react together and how the cell membrane is formed to give the cell its structure and stability. Many of the functional groups commonly used in organic chemistry are also frequently seen in nature: methyl (CH3), hydroxyl (OH), carboxyl (COOH), and amino (NH2) recur repeatedly in biology. The small organic molecules found in the cell generally contain up to 30 atoms and have many uses in the cell, such as intermediates for deriving energy from food and units to build polymers which comprise the majority of the cells composition.
The synthesis and breakdown of biopolymers follow a discrete sequence of chemical changes and follow definite rules. As a result, many of the biological compounds that make up the cell are chemically related and can be broadly classified into four major families of small organic molecules: the simple sugars, the fatty acids, the amino acids, and the nucleotides. Sugars are the food molecules of the cell; broken down to create chemical energy. Fatty acids play an important role as the main component of the cell membrane, and amino acids and nucleotides are the sub-units of two crucial groups of biopolymers: proteins and nucleic acids, respectively.
Biological molecules may consist of many thousands, or even millions of these sub-units to produce structures where each atom is precisely linked into a specific spatial arrangement; imperative to determine the macromolecule’s properties, which in turn governs their specific function in the cell. The specific sequence by which these sub-units, be it amino acids or nucleotides, are organised carries specific information and generates a biological message that can be “read” through interactions with other molecules. This is how biological macromolecules perform their function: by interacting with the appropriate molecular counterparts, governed by specific intermolecular forces that exist between them, macromolecules can perform roles in the cell such as catalysing chemical transformations, assembly into multi-molecular structures, generate motion, and, most fundamental, storing hereditary information.
The cell is an aqueous environment, where water comprises 70% of the total mass. The remainder is mainly due to macromolecules. These are assembled from their monomer sub-units through particular biochemical mechanisms which specifically control the sequence of the monomers added to the end of the polymer chain as well as determining the appropriate time to terminate the sequence. The macromolecular chain is linked by covalent bonds, which are strong enough to preserve the sequence for a long period of time. This precise sequence dictates the information contained within the macromolecule, but utilization of the information is controlled through non-covalent interactions. These are much weaker bonds which exist between the polar functional groups between different molecules or different parts of the same molecule. These electrostatic interactions govern the three-dimentsional shape of a macromolecule and, therefore, how it will interact with other molecules.
These interactions singularly are too weak to withstand thermal motions; hence, macromolecular structures are held in place by multiple non-covalent interactions. For this to occur, spatial arrangements of the atoms must be precisely matched for a strong interaction. For example, in the case of hydrogen bonding, which is a particular kind of non-covalent interaction between a lone pair of electrons on a sufficiently electronegative atom, namely nitrogen, oxygen, or fluorine, and the electron deficient hydrogen atom bonded to the aforementioned elements. The donated lone pair of electrons must be in the correct orientation relative to the accepting hydrogen atom of the other molecule/other part of the same molecule to satisfy the directionality of the intermolecular force. In biochemistry, usually multiples of these interactions are required between molecules, which leads to the need for interacting molecules to...
Inhaltsverzeichnis
Zitierstile für Miraculous Medicines and the Chemistry of Drug Design
APA 6 Citation
Keighley, N. (2020). Miraculous Medicines and the Chemistry of Drug Design (1st ed.). CRC Press. Retrieved from https://www.perlego.com/book/2038241/miraculous-medicines-and-the-chemistry-of-drug-design-pdf (Original work published 2020)
Chicago Citation
Keighley, Nathan. (2020) 2020. Miraculous Medicines and the Chemistry of Drug Design. 1st ed. CRC Press. https://www.perlego.com/book/2038241/miraculous-medicines-and-the-chemistry-of-drug-design-pdf.
Harvard Citation
Keighley, N. (2020) Miraculous Medicines and the Chemistry of Drug Design. 1st edn. CRC Press. Available at: https://www.perlego.com/book/2038241/miraculous-medicines-and-the-chemistry-of-drug-design-pdf (Accessed: 15 October 2022).
MLA 7 Citation
Keighley, Nathan. Miraculous Medicines and the Chemistry of Drug Design. 1st ed. CRC Press, 2020. Web. 15 Oct. 2022.