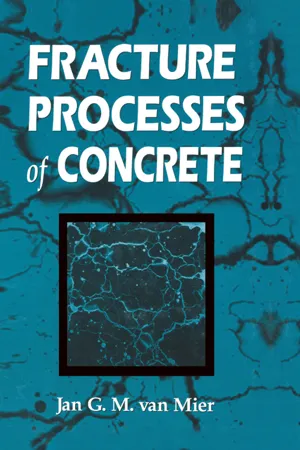
eBook - ePub
Fracture Processes of Concrete
Jan G.M. van Mier
This is a test
Partager le livre
- 464 pages
- English
- ePUB (adapté aux mobiles)
- Disponible sur iOS et Android
eBook - ePub
Fracture Processes of Concrete
Jan G.M. van Mier
Détails du livre
Aperçu du livre
Table des matiĂšres
Citations
Ă propos de ce livre
Despite tremendous advances made in fracture mechanics of concrete in recent years, very little information has been available on the nature of fracture processes and on reliable test methods for determining parameters for the different models. Moreover, most texts on this topic discuss numerical modeling but fail to consider experimentation. This book fills these gaps and synthesizes progress in the field in a simple, straightforward manner geared to practical applications.
Foire aux questions
Comment puis-je résilier mon abonnement ?
Il vous suffit de vous rendre dans la section compte dans paramĂštres et de cliquer sur « RĂ©silier lâabonnement ». Câest aussi simple que cela ! Une fois que vous aurez rĂ©siliĂ© votre abonnement, il restera actif pour le reste de la pĂ©riode pour laquelle vous avez payĂ©. DĂ©couvrez-en plus ici.
Puis-je / comment puis-je télécharger des livres ?
Pour le moment, tous nos livres en format ePub adaptĂ©s aux mobiles peuvent ĂȘtre tĂ©lĂ©chargĂ©s via lâapplication. La plupart de nos PDF sont Ă©galement disponibles en tĂ©lĂ©chargement et les autres seront tĂ©lĂ©chargeables trĂšs prochainement. DĂ©couvrez-en plus ici.
Quelle est la différence entre les formules tarifaires ?
Les deux abonnements vous donnent un accĂšs complet Ă la bibliothĂšque et Ă toutes les fonctionnalitĂ©s de Perlego. Les seules diffĂ©rences sont les tarifs ainsi que la pĂ©riode dâabonnement : avec lâabonnement annuel, vous Ă©conomiserez environ 30 % par rapport Ă 12 mois dâabonnement mensuel.
Quâest-ce que Perlego ?
Nous sommes un service dâabonnement Ă des ouvrages universitaires en ligne, oĂč vous pouvez accĂ©der Ă toute une bibliothĂšque pour un prix infĂ©rieur Ă celui dâun seul livre par mois. Avec plus dâun million de livres sur plus de 1 000 sujets, nous avons ce quâil vous faut ! DĂ©couvrez-en plus ici.
Prenez-vous en charge la synthÚse vocale ?
Recherchez le symbole Ăcouter sur votre prochain livre pour voir si vous pouvez lâĂ©couter. Lâoutil Ăcouter lit le texte Ă haute voix pour vous, en surlignant le passage qui est en cours de lecture. Vous pouvez le mettre sur pause, lâaccĂ©lĂ©rer ou le ralentir. DĂ©couvrez-en plus ici.
Est-ce que Fracture Processes of Concrete est un PDF/ePUB en ligne ?
Oui, vous pouvez accĂ©der Ă Fracture Processes of Concrete par Jan G.M. van Mier en format PDF et/ou ePUB ainsi quâĂ dâautres livres populaires dans Technik & Maschinenbau et Bauingenieurwesen. Nous disposons de plus dâun million dâouvrages Ă dĂ©couvrir dans notre catalogue.
Informations
Chapter 1
INTRODUCTION: SETTING THE STAGE
1.1 CRACKING IN CONCRETE AND CONCRETE STRUCTURES
Cracks are everywhere around us. Any brittle material shows cracks at some stage of the life cycle. Humans have always tried to understand the mechanisms behind the growth of cracks and, through the decades, in particular after the pioneering work of Griffith1* on the brittle growth of cracks, much research has been directed towards a better and deeper understanding of the observed phenomena. Much of the research has traditionally focused on brittle materials like cast iron and glass. Interest in fracture of ductile metals emerged after Dugdale2 and Barenblatt3 developed a plastic crack tip model. All such models, including the very recent efforts to come to a unified approach to describe fracture phenomena in brittle disordered materials (concrete, rock and ceramics) rely heavily on experimental observations. In a time when numerical simulation is the key word in many research proposals, the role of experimentation seems however more and more under pressure and largely neglected. Of course, it is possible to keep clean hands sitting behind a computer screen. But, do the materials behave as we think they should behave? Traditionally, the role of testing has been much larger in structural and materials engineering. The analytical tools available to the engineer did not allow full computation of the behaviour of structures, and many educated guesses (engineering judgement) were necessary before new structural shapes were tried, or larger spans were introduced. With the introduction of numerical methods in engineering in the 1970s, many people believed that âpredictionâ of structural and material behaviour would be a matter of time. The only criterion seemed that new faster computational facilities would become available. These developments have, in other areas of science, led to speculations that eventually consciousness (a topic that could not be handled using traditional scientific methods) can be explained from numerical analogies too, e.g. Dennett.4
As mentioned, cracks are everywhere. In normal reinforced concrete structures cracking must occur to allow for the transfer of tensile stresses from the concrete to the steel. Such cracks, however, impair the durability and life span of the material and structures. Examples where durability becomes a leading issue is with the development of large off-shore structures. Examples are artificial islands for the recovery of oil, large bridges spanning sea arms like the Store BĂŠlt bridge in Denmark, the Eastern Scheldt Barrier which was constructed in The Netherlands to protect the low lands from flooding during severe autumn and winter tempests, or high-rise buildings (Figure 1.1). Structures in a marine environment are particularly susceptible to the intrusion of chlorides and sulphates from the sea-water. This may lead to corrosion of the prestressing or reinforcing steel, or to swelling of aggregates containing reactive silica. Not only is chemical attack to be considered important, but physical and mechanical loadings may cause cracking in building and civil engineering structures as well. Several strategies can be followed to try to avoid crack growth. One may try to avoid cracking completely, for example, by allowing moderate stress levels only. Needless to say, this will not lead to very practical or very economical structures. Other solutions aim at reducing the crack width by properly designing the reinforcement details, like diameter, concrete cover, etc. Again others try to solve the durability and structural problems by selecting non-corrosive reinforcement, but then other problems may have to be solved, for example the bond between the alternative reinforcement and the concrete. These are all issues that will not be dealt with in this book. Rather, we will try to come to a better and more profound understanding of crack nucleation and growth in concrete materials and structures. One of the most important issues in fracture mechanics is that the behaviour of the material and structure cannot be separated. Quite curiously, this strong interdependence between material response and structural behaviour seems to be grossly neglected. Moreover, the level of observation is very important, as will become clear in the book.
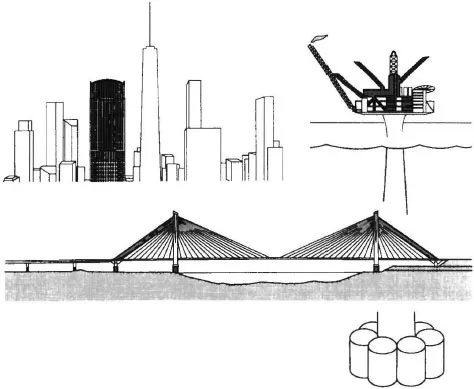
Figure 1.1 Examples of advanced, modern (reinforced) concrete structures.
Upon considering basic fracture mechanisms of concrete under mechanical loading â which is one of the important limitations made in this book â one should address tensile, shear, compression and multiaxial states of stress, including any possible path dependency, rate effects and creep. Furthermore, one should address chemicolmechanical and physico/mechanical interactions. However, for a single person to comprehend all these loading cases, as well as the behaviour of structures and materials in sufficient detail, becomes more and more problematic as researchers tend to limit their activities to areas of limited scope. In the book we will aim at a much improved interaction and collaboration between different disciplines. In order to demonstrate that cooperation between disciplines is important in future research, many examples are given which show that an interaction between materials science, fracture testing and computational modelling is essential.
Interactions between disciplines, mechanical loading with a limited number of side-steps to physico/mechanical interactions, are dealt with in the book. In an effort to elucidate fracture processes in complex heterogeneous materials like concrete, the definition of the level at which the observations and models are made is of extreme importance. Therefore, in Section 1.3 we will clearly define the various levels of observation that will be used in the book. But before doing so, a very short and limited introduction of fracture criteria that have been introduced over the years, will be given in Section 1.2.
1.2 LIMIT THEORIES OF MATERIALS AND STRUCTURES
Structural engineering is generally based on strength-of-materials theories. It is assumed that failure occurs as soon as a limit strength is exceeded, and for a description of the material used in the structure, a simple determination of the strength of a material sample is considered to be sufficient. In Figure 1.2, this behaviour is indicated by means of an elastic-purely brittle stress-strain law. The determinations of the strength of the material under a wide variety of mechanical loads like uniaxial tension, uniaxial compression, and all kinds of multiaxial loads will suffice in this simplified view.
The strength could simply be determined by increasing the dead-load on a sample, and no sophisticated equipment is needed. For materials like rock, concrete and glass, in general, sudden failure was observed, but for many other materials like metals, it was found that larger deformations at a constant stress-level were needed. Ductile behaviour is observed, which is shown in Figure 1.2 as well. A perfect elastic-perfectly plastic stress-strain diagram is shown. Also, in the figure it is indicated that upon unloading not all deformation is recovered, but large irreversible deformations occur. New technical possibilities allowed for the measurement of deformations. Such measurements eventually demonstrated that in the beginning of the plastic plateau in the diagram of Figure 1.2, deformations are uniformly distributed in the sample, but later on they were localised in a very narrow zone of the test specimen. Moreover, it was also found that the linear elastic stress-strain behaviour up till reaching a peak stress-level, was more based on assumptions and preferred behaviour than on reality. Again, further developments of testing techniques, more important than anything else, led to the insight that some materials that were previously assumed to be brittle showed a distinct life after the peak stress level was reached. The stress-strain behaviour is shown in Figure 1.2c. Dedicated electronics nowadays allow for unrestricted testing in this so-called post-peak regime (or softening regime), and in the past two decades, much progress has been made in a further understanding of this life beyond peak.
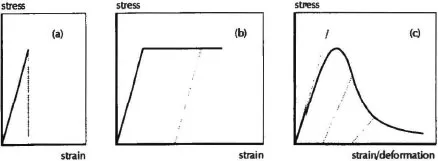
Figure 1.2 Basic behaviour laws for materials: (a) elastic-brittle, (b) elasto-plastic, and (c) softening.
Theoretically one can compute the ultimate strength of materials from fundamental interactions between atoms, or even from interactions between sub-atomic particles. It was the great achievement of Griffith to demonstrate, and to indicate ways to tackle the problem theoretically, that the ideal strength of materials can never be reached because stress concentrations due to impurities will cause the material to fail at a substantial lower level of stress. Later, to be more specific in the 1970s, this led to the formulation of a dedicated model for concrete fracture by Hillerborg et al.5
In all observations that are fundamental to mechanical behaviour laws (constitutive models) for materials, the material is considered as a black box. It is assumed that the material behaves as a continuum, i.e., as a material that has the same properties in every point. The fact that different structural features can be found in a material at different levels of observation leads to the idea that much of the behaviour observed at one level can be explained in terms of the material structure (and changes therein) at a lower level. In fact, one example was already given. Reinforced concrete can be considered as a continuum material with no internal structure, as for example proposed by Vecchio and Collins.6 However, more widespread is the approach where reinforced concrete is regarded as a composite of the two basic materials steel and concrete. In the next section we will further explore the effect of the level of observation.
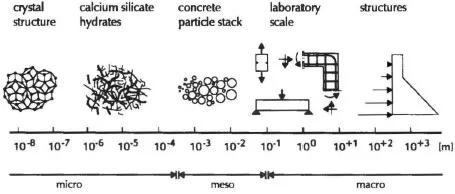
Figure 1.3 From atomic structure to large-scale building and civil engineering structures, exemplifying the various scales of observation that must be considered when studying materials and structures.
One last remark should be made. In all âclassicalâ models, a limit strength or stress or even a critical stress intensity factor is proposed as a fundamental criterion leading to macroscopic failure. In the last decades, energy methods have been proposed as well. It should be mentioned here that strength, stress and energy are man-made quantities. In reality, a material or structure can experience deformations only.
1.3 DIFFERENT LEVELS OF OBSERVATION
Most of the classical limit models are based on the assumption that the material can be represented as a continuum. The behaviour is described independent of the structure of the material. The only geometrical ingredients are formed by the size and shape of the structure that was considered. For any material it is possible to construct a diagram as done in Figure 1.3 for concrete. Concrete is a multi-scale material. Cement, sand, aggregates, water, and sometimes a number of other additives are mixed together and harden to form a solid material. The smallest structural feature in Figure 1.3 is the atomic structure of the cement and aggregates. We are observing the material at the nanometer scale (10â9 m). At the micrometer scale (10â6 m) the individual cement grains are distinguished. Before hardening, the unhydrated cement grains can be observed; in the hardened state calcium silicate hydrates and calcium-hydroxide are visible. The complex pore structure becomes visible as well. Upon increasing the scale to 10â3 m, individual sand and aggregate particles can be distinguished. Larger pores can be found as well, and the interaction between the aggregate particles and the cement matrix is one of the essential features at this level of observation. At 10+1 m, we have reached the scale at which laboratory scale (mechanical) experiments on concrete are carried out. No internal structure is recognised at this level, and the material is assumed to have identical properties in each point of the specimen or structure. At even larger scales, i.e., up to 10+2 to 10+3 m, we reach the scale of building and civil engineering structures. As mentioned before, the structures can be considered at the macro-level where reinforced concrete is considered as a continuum material, or alternatively, one might recognize some internal structure, more specifically the structure formed by the steel reinforcement. All these levels are represented in Figure 1.3. In engineering and science of concrete materials and structures, research has focused mainly on three distinct levels, namely the micro-, meso- and macro-level. By adopting familiar ideas from materials science, Wittmann7 was the first to recognise that this so-called three-level approach could be a useful guide-line for research on concrete materials and structures. The three levels of observation are shown again in Figure 1.4, distinguishing the structural features that are important at each level. At the micro-level, the internal structure of cement and hardened cement paste is the most important structural feature. At the meso-level, the particle structure is most important. The heterogeneous nature of the material is the reason that local stress-concentrations appear, much in line with the earlier observation of Griffith that pores and impurities in materials set a limit to the strength of the material. At the macro-level, which is essentially the level at which structural engineers are working, no internal material structure is recognised, except for reinforced concrete where the reinforcement structure is normally taken into account.
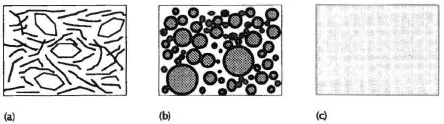
Figure 1.4 Definition of (a) micro-, (b) meso-, and (c) macro-level for cement and concrete.
In the three-level approach, it is generally assumed that behaviour at one level can ...